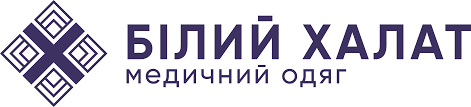
Знижка - 10% на весь медичний одяг по промокоду HALAT5
After studying this chapter, you will be able to:
The smallest, most fundamental material components of the human body are basic chemical elements. In fact, chemicals called nucleotide bases are the foundation of the genetic code with the instructions on how to build and maintain the human body from conception through old age. There are about three billion of these base pairs in human DNA.
Human chemistry includes organic molecules (carbon-based) and biochemicals (those produced by the body). Human chemistry also includes elements. In fact, life cannot exist without many of the elements that are part of the earth. All of the elements that contribute to chemical reactions, to the transformation of energy, and to electrical activity and muscle contraction—elements that include phosphorus, carbon, sodium, and calcium, to name a few—originated in stars.
These elements, in turn, can form both the inorganic and organic chemical compounds important to life, including, water, glucose, and proteins. This chapter begins by examining elements and how the structures of atoms, the basic units of matter, determine the characteristics of elements by the number of protons, neutrons, and electrons in the atoms. The chapter then builds the framework of life from there.
By the end of this section, you will be able to:
The substance of the universe—from a grain of sand to a star—is called matter. Scientists define matter as anything that occupies space and has mass. An object’s mass and its weight are related concepts, but not quite the same. An object’s mass is the amount of matter contained in the object, and is the same whether that object is on Earth or in the zero-gravity environment of outer space. An object’s weight, on the other hand, is its mass as affected by the pull of gravity. An object’s weight is greater where the pull of gravity is stronger than where the gravity is less strong. For example, an object of a certain mass weighs less on the moon than it does on Earth because the gravity of the moon is less than that of Earth. In other words, weight is variable, and is influenced by gravity. A piece of cheese that weighs a pound on Earth weighs only a few ounces on the moon.
Elements and Compounds
All matter in the natural world is composed of one or more of the 92 fundamental substances called elements. An element is a pure substance that is distinguished from all other matter by the fact that it cannot be created or broken down by ordinary chemical means. While your body can assemble many of the chemical compounds needed for life from their constituent elements, it cannot make elements. They must come from the environment. A familiar example of an element that you must take in is calcium (Ca++). Calcium is essential to the human body; it is absorbed and used for a number of processes, including strengthening bones. When you consume dairy products your digestive system breaks down the food into components small enough to cross into the bloodstream. Among these is calcium, which, because it is an element, cannot be broken down further. The elemental calcium in cheese, therefore, is the same as the calcium that forms your bones. Some other elements you might be familiar with are oxygen, sodium, and iron. The elements in the human body are shown in Figure 2.1.1, beginning with the most abundant: oxygen (O), carbon (C), hydrogen (H), and nitrogen (N). Each element’s name can be replaced by a one- or two-letter symbol; you will become familiar with some of these during this course. All the elements in your body are derived from the foods you eat and the air you breathe.
In nature, elements rarely occur alone. Instead, they combine to form compounds. A compound is a substance composed of two or more elements joined by chemical bonds. For example, the compound glucose is an important body fuel. It is always composed of the same three elements: carbon, hydrogen, and oxygen. Moreover, the elements that make up any given compound always occur in the same relative amounts. In glucose, there are always six carbon and six oxygen units for every twelve hydrogen units. But what, exactly, are these “units” of elements?
Atoms and Subatomic Particles
An atom is the smallest quantity of an element that retains the unique properties of that element. In other words, an atom of hydrogen is a unit of hydrogen—the smallest amount of hydrogen that can exist. As you might guess, atoms are almost unfathomably small. The period at the end of this sentence is millions of atoms wide.
Atomic Structure and Energy
Atoms are made up of even smaller subatomic particles, which include three important types: the proton, neutron, and electron. The number of positively-charged protons and non-charged (“neutral”) neutrons, gives mass to the atom, and the number of each in the nucleus of the atom determines the element. The number of negatively-charged electrons that “spin” around the nucleus at close to the speed of light equals the number of protons. An electron has about 1/2000th the mass of a proton or neutron.
Figure 2.1.2 shows two models that can help you imagine the structure of an atom—in this case, helium (He). In the planetary model, helium’s two electrons are shown circling the nucleus in a fixed orbit depicted as a ring. Although this model is helpful in visualizing atomic structure, in reality, electrons do not travel in fixed orbits, but whiz around the nucleus erratically in a so-called electron cloud.
An atom’s protons and electrons carry electrical charges. Protons, with their positive charge, are designated p+. Electrons, which have a negative charge, are designated e–. An atom’s neutrons have no charge: they are electrically neutral. Just as a magnet sticks to a steel refrigerator because their opposite charges attract, the positively charged protons attract the negatively charged electrons. This mutual attraction gives the atom some structural stability. The attraction by the positively charged nucleus helps keep electrons from straying far. The number of protons and electrons within a neutral atom are equal, thus, the atom’s overall charge is balanced.
Atomic Number and Mass Number
An atom of carbon is unique to carbon, but a proton of carbon is not. One proton is the same as another, whether it is found in an atom of carbon, sodium (Na), or iron (Fe). The same is true for neutrons and electrons. So, what gives an element its distinctive properties—what makes carbon so different from sodium or iron? The answer is the unique quantity of protons each contains. Carbon by definition is an element whose atoms contain six protons. No other element has exactly six protons in its atoms. Moreover, all atoms of carbon, whether found in your liver or in a lump of coal, contain six protons. Thus, the atomic number, which is the number of protons in the nucleus of the atom, identifies the element. Since an atom usually has the same number of electrons as protons, the atomic number identifies the usual number of electrons as well.
In their most common form, many elements also contain the same number of neutrons as protons. The most common form of carbon, for example, has six neutrons as well as six protons, for a total of 12 subatomic particles in its nucleus. An element’s mass number is the sum of the number of protons and neutrons in its nucleus. So the most common form of carbon’s mass number is 12. Electrons have so little mass that they do not appreciably contribute to the mass of an atom. Carbon is a relatively light element; Uranium (U), in contrast, has a mass number of 238 and is referred to as a heavy metal. Its atomic number is 92 (it has 92 protons) but it contains 146 neutrons; it has the most mass of all the naturally occurring elements.
The periodic table of the elements, shown in Figure 2.1.3, is a chart identifying the 92 elements found in nature, as well as several larger, unstable elements discovered experimentally. The elements are arranged in order of their atomic number, with hydrogen and helium at the top of the table, and the more massive elements below. The periodic table is a useful device because for each element, it identifies the chemical symbol, the atomic number, and the mass number, while organizing elements according to their propensity to react with other elements. The number of protons and electrons in an element are equal. The number of protons and neutrons may be equal for some elements, but are not equal for all.
Isotopes
Although each element has a unique number of protons, it can exist as different isotopes. An isotope is one of the different forms of an element, distinguished from one another by different numbers of neutrons. The standard isotope of carbon is 12C, commonly called carbon twelve. 12C has six protons and six neutrons, for a mass number of twelve. All of the isotopes of carbon have the same number of protons; therefore, 13C has seven neutrons, and 14C has eight neutrons. The different isotopes of an element can also be indicated with the mass number hyphenated (for example, C-12 instead of 12C). Hydrogen has three common isotopes, shown in Figure 2.1.4.
An isotope that contains more than the usual number of neutrons is referred to as a heavy isotope. An example is 14C. Heavy isotopes tend to be unstable, and unstable isotopes are radioactive. A radioactive isotope is an isotope whose nucleus readily decays, giving off subatomic particles and electromagnetic energy. Different radioactive isotopes (also called radioisotopes) differ in their half-life, the time it takes for half of any size sample of an isotope to decay. For example, the half-life of tritium—a radioisotope of hydrogen—is about 12 years, indicating it takes 12 years for half of the tritium nuclei in a sample to decay. Excessive exposure to radioactive isotopes can damage human cells and even cause cancer and birth defects, but when exposure is controlled, some radioactive isotopes can be useful in medicine. For more information, see the Career Connections.
The controlled use of radioisotopes has advanced medical diagnosis and treatment of disease. Interventional radiologists are physicians who treat disease by using minimally invasive techniques involving radiation. Many conditions that could once only be treated with a lengthy and traumatic operation can now be treated non-surgically, reducing the cost, pain, length of hospital stay, and recovery time for patients. For example, in the past, the only options for a patient with one or more tumors in the liver were surgery and chemotherapy (the administration of drugs to treat cancer).
Some liver tumors, however, are difficult to access surgically, and others could require the surgeon to remove too much of the liver; chemotherapy is highly toxic to the liver, and certain tumors do not respond well to it. In some such cases, an interventional radiologist can treat the tumors by disrupting their blood supply, which they need if they are to continue to grow. In this procedure, called radioembolization, the radiologist accesses the liver with a fine needle, threaded through one of the patient’s blood vessels. The radiologist then inserts tiny radioactive “seeds” into the blood vessels that supply the tumors. In the days and weeks following the procedure, the radiation emitted from the seeds destroys the vessels and directly kills the tumor cells in the vicinity of the treatment.
Radioisotopes emit subatomic particles that can be detected and tracked by imaging technologies. One of the most advanced uses of radioisotopes in medicine is the positron emission tomography (PET) scanner, which detects the activity in the body of a very small injection of radioactive glucose, the simple sugar that cells use for energy. The PET camera shows the medical team which of the patient’s tissues are taking up the most glucose. Thus, the most metabolically active tissues show up as bright “hot spots” on the images (Figure 2.1.5). PET can reveal some cancerous masses because cancer cells consume glucose at a high rate to fuel their rapid reproduction.
The Behavior of Electrons
In the human body, atoms do not exist as independent entities. Rather, they are constantly reacting with other atoms to form and to break down more complex substances. To fully understand anatomy and physiology you must grasp how atoms participate in such reactions. The key is understanding the behavior of electrons.
Although electrons do not follow rigid orbits a set distance away from the atom’s nucleus, they do tend to stay within certain regions of space called electron shells. An electron shell is a layer of electrons that encircle the nucleus at a distinct energy level.
The atoms of the elements found in the human body have from one to five electron shells, and all electron shells hold eight electrons except the first shell, which can only hold two. This configuration of electron shells is the same for all atoms. The precise number of shells depends on the number of electrons in the atom. Hydrogen and helium have just one and two electrons, respectively. If you take a look at the periodic table of the elements, you will notice that hydrogen and helium are placed alone on either sides of the top row; they are the only elements that have just one electron shell (Figure 2.1.6). A second shell is necessary to hold the electrons in all elements larger than hydrogen and helium.
Lithium (Li), whose atomic number is 3, has three electrons. Two of these fill the first electron shell, and the third spills over into a second shell. The second electron shell can accommodate as many as eight electrons. Carbon, with its six electrons, entirely fills its first shell, and half-fills its second. With ten electrons, neon (Ne) entirely fills its two electron shells. Again, a look at the periodic table reveals that all of the elements in the second row, from lithium to neon, have just two electron shells. Atoms with more than ten electrons require more than two shells. These elements occupy the third and subsequent rows of the periodic table.
The factor that most strongly governs the tendency of an atom to participate in chemical reactions is the number of electrons in its valence shell. A valence shell is an atom’s outermost electron shell. If the valence shell is full, the atom is stable, meaning its electrons are unlikely to be pulled away from the nucleus by the electrical charge of other atoms. If the valence shell is not full, the atom is reactive, meaning it will tend to react with other atoms in ways that make the valence shell full. Consider hydrogen, with its one electron only half-filling its valence shell. This single electron is likely to be drawn into relationships with the atoms of other elements, so that hydrogen’s single valence shell can be stabilized.
All atoms (except hydrogen and helium with their single electron shells) are most stable when there are exactly eight electrons in their valence shell. This principle is referred to as the octet rule, and it states that an atom will give up, gain, or share electrons with another atom so that it ends up with eight electrons in its own valence shell. For example, oxygen, with six electrons in its valence shell, is likely to react with other atoms in a way that results in the addition of two electrons to oxygen’s valence shell, bringing the number to eight. When two hydrogen atoms each share their single electron with oxygen, covalent bonds are formed, resulting in a molecule of water, H2O.
In nature, atoms of one element tend to join with atoms of other elements in characteristic ways. For example, carbon commonly fills its valence shell by linking up with four atoms of hydrogen. In so doing, the two elements form the simplest of organic molecules—methane—which also is one of the most abundant and stable carbon-containing compounds on Earth. As stated above, another example is water; oxygen needs two electrons to fill its valence shell. It commonly interacts with two atoms of hydrogen, forming H2O. Incidentally, the name “hydrogen” reflects its contribution to water (hydro- = “water”; -gen = “maker”). Thus, hydrogen is the “water maker.”
The human body is composed of elements, the most abundant of which are oxygen (O), carbon (C), hydrogen (H) and nitrogen (N). You obtain these elements from the foods you eat and the air you breathe. The smallest unit of an element that retains all of the properties of that element is an atom. Atoms themselves contain many subatomic particles, the three most important of which are protons, neutrons, and electrons. These particles do not vary in quality from one element to another; rather, what gives an element its distinctive identification is the quantity of its protons, called its atomic number. Protons and neutrons contribute nearly all of an atom’s mass; the number of protons and neutrons is an element’s mass number. Heavier and lighter versions of the same element can occur in nature because these versions have different numbers of neutrons. Different versions of an element are called isotopes.
The tendency of an atom to be stable or to react readily with other atoms is largely due to the behavior of the electrons within the atom’s outermost electron shell, called its valence shell. Helium, as well as larger atoms with eight electrons in their valence shell, is unlikely to participate in chemical reactions because they are stable. All other atoms tend to accept, donate, or share electrons in a process that brings the electrons in their valence shell to eight (or in the case of hydrogen, to two).
By the end of this section, you will be able to:
Atoms separated by a great distance cannot link; rather, they must come close enough for the electrons in their valence shells to interact. But do atoms ever actually touch one another? Most physicists would say no, because the negatively charged electrons in their valence shells repel one another. No force within the human body—or anywhere in the natural world—is strong enough to overcome this electrical repulsion. So when you read about atoms linking together or colliding, bear in mind that the atoms are not merging in a physical sense.
Instead, atoms link by forming a chemical bond. A bond is a weak or strong electrical attraction that holds atoms in the same vicinity. The new grouping is typically more stable—less likely to react again—than its component atoms were when they were separate. A more or less stable grouping of two or more atoms held together by chemical bonds is called a molecule. The bonded atoms may be of the same element, as in the case of H2, which is called molecular hydrogen or hydrogen gas. When a molecule is made up of two or more atoms of different elements, it is called a chemical compound. A unit of water, or H2O, is a compound, as is a single molecule of the gas methane, or CH4.
Three types of chemical bonds are important in human physiology, because they hold together substances that are used by the body for critical aspects of homeostasis, signaling, and energy production, to name just a few important processes. These are ionic bonds, covalent bonds, and hydrogen bonds.
Ions and Ionic Bonds
Recall that an atom typically has the same number of positively charged protons and negatively charged electrons. As long as this situation remains, the atom is electrically neutral. When an atom participates in a chemical reaction that results in the donation or acceptance of one or more electrons, the atom will then become positively or negatively charged. This happens frequently for most atoms in order to have a full valence shell, as described previously. This can happen either by gaining electrons to fill a shell that is more than half-full, or by giving away electrons to empty a shell that is less than half-full, thereby leaving the next smaller electron shell as the new, full, valence shell. An atom that has an electrical charge—whether positive or negative—is an ion.
Potassium (K), for instance, is an important element in all body cells. Its atomic number is 19 and it has just one electron in its valence shell. This characteristic makes potassium highly likely to participate in chemical reactions in which it donates one electron (it is easier for potassium to donate one electron than to gain seven electrons). The loss will cause the positive charge of potassium’s protons to be more influential than the negative charge of potassium’s electrons. In other words, the resulting potassium ion will be slightly positive. A potassium ion is written K+, indicating that it has lost a single electron. A positively charged ion is known as a cation.
Now consider fluorine (F), a component of bones and teeth. Its atomic number is nine and it has seven electrons in its valence shell. Thus, it is highly likely to bond with other atoms in such a way that fluorine accepts one electron (it is easier for fluorine to gain one electron than to donate seven electrons). When it does, its electrons will outnumber its protons by one and it will have an overall negative charge. The ionized form of fluorine is called fluoride, and is written as F–. A negatively charged ion is known as an anion.
Atoms that have more than one electron to donate or accept will end up with stronger positive or negative charges. A cation that has donated two electrons has a net charge of +2. Using magnesium (Mg) as an example, this can be written as Mg++ or Mg2+. An anion that has accepted two electrons has a net charge of –2. The ionic form of selenium (Se), for example, is typically written Se2–.
The opposite charges of cations and anions exert a moderately strong mutual attraction that keeps the atoms in close proximity forming an ionic bond. An ionic bond is an ongoing, close association between ions of opposite charge. The table salt you sprinkle on your food owes its existence to ionic bonding. As shown in Figure 2.2.1, sodium commonly donates an electron to chlorine, becoming the cation Na+. When chlorine accepts the electron, it becomes the chloride anion, Cl–. With their opposing charges, these two ions strongly attract each other.
Water is an essential component of life because it is able to break the ionic bonds in salts to free the ions. In fact, in biological fluids, most individual atoms exist as ions. These dissolved ions produce electrical charges within the body. The behavior of these ions produces the tracings of heart and brain function observed as waves on an electrocardiogram (EKG or ECG) or an electroencephalogram (EEG). The electrical activity that derives from the interactions of the charged ions is why they are also called electrolytes.
Covalent Bonds
Unlike ionic bonds formed by the attraction between a cation’s positive charge and an anion’s negative charge, molecules formed by a covalent bond which share electrons in a mutually stabilizing relationship. Like next-door neighbors whose kids hang out first at one home and then at the other, the atoms do not lose or gain electrons permanently. Instead, the electrons move back and forth between the elements. Because of the close sharing of pairs of electrons (one electron from each of two atoms), covalent bonds are stronger than ionic bonds.
Nonpolar Covalent Bonds
Figure 2.2.2 shows several common types of covalent bonds. Notice that the two covalently bonded atoms typically share just one or two electron pairs, though larger sharings are possible. The important concept to take from this is that in covalent bonds, electrons in the outermost valence shell are shared to fill the valence shells of both atoms, ultimately stabilizing both of the atoms involved. In a single covalent bond, a single electron is shared between two atoms, while in a double covalent bond, two pairs of electrons are shared between two atoms. There are even triple covalent bonds, where three atoms are shared.
You can see that the covalent bonds shown in Figure 2.2.2 are balanced. The sharing of the negative electrons is relatively equal, as is the electrical pull of the positive protons in the nucleus of the atoms involved. This is why covalently bonded molecules that are electrically balanced in this way are described as nonpolar; that is, no region of the molecule is either more positive or more negative than any other.
Polar Covalent Bonds
Groups of legislators with completely opposite views on a particular issue are often described as “polarized” by news writers. In chemistry, a polar molecule is a molecule that contains regions that have opposite electrical charges. Polar molecules occur when atoms share electrons unequally, in polar covalent bonds.
The most familiar example of a polar molecule is water (Figure 2.2.3). The molecule has three parts: one atom of oxygen, the nucleus of which contains eight protons, and two hydrogen atoms, whose nuclei each contain only one proton. Since every proton exerts an identical positive charge, a nucleus that contains eight protons exerts a charge eight times greater than a nucleus that contains one proton. This means that the negatively charged electrons present in the water molecule are more strongly attracted to the oxygen nucleus than to the hydrogen nuclei. Each hydrogen atom’s single negative electron, therefore, migrates toward the oxygen atom, making the oxygen end of their bond slightly more negative than the hydrogen end of their bond.
What is true for the bonds is true for the water molecule as a whole; that is, the oxygen region has a slightly negative charge and the regions of the hydrogen atoms have a slightly positive charge. These charges are often referred to as “partial charges” because the strength of the charge is less than one full electron, as would occur in an ionic bond. As shown in Figure 2.2.3, regions of weak polarity are indicated with the Greek letter delta (∂) and a plus (+) or minus (–) sign.
Even though a single water molecule is unimaginably tiny, it has mass, and the opposing electrical charges on the molecule pull that mass in such a way that it creates a shape somewhat like a triangular tent (see Figure 2.2.3b). This dipole, with the positive charges at one end formed by the hydrogen atoms at the “bottom” of the tent and the negative charge at the opposite end (the oxygen atom at the “top” of the tent) makes the charged regions highly likely to interact with charged regions of other polar molecules. For human physiology, the resulting bond, formed by water, is one of the most important—the hydrogen bond.
Hydrogen Bonds
A hydrogen bond is formed when a weakly positive hydrogen atom already bonded to one electronegative atom (for example, the oxygen in the water molecule) is attracted to another electronegative atom from another molecule. In other words, hydrogen bonds always include hydrogen that is already part of a polar molecule.
The most common example of hydrogen bonding in the natural world occurs between molecules of water. It happens before your eyes whenever two raindrops merge into a larger bead, or a creek spills into a river. Hydrogen bonding occurs because the weakly negative oxygen atom in one water molecule is attracted to the weakly positive hydrogen atoms of two other water molecules (Figure 2.2.4).
Notice that the bonds occur between the weakly positive charge on the hydrogen atoms and the weakly negative charge on the oxygen atoms. Hydrogen bonds are relatively weak, and therefore are indicated with a dotted (rather than a solid) line.
Water molecules also strongly attract other types of charged molecules as well as ions. This explains why “table salt,” for example, actually is a molecule called a “salt” in chemistry; it consists of equal numbers of positively-charged sodium (Na+) and negatively-charged chloride (Cl–), dissolves so readily in water, in this case, forming dipole-ion bonds between the water and the electrically-charged ions (electrolytes). Water molecules also repel molecules with nonpolar covalent bonds, like fats, lipids, and oils. You can demonstrate this with a simple kitchen experiment: pour a teaspoon of vegetable oil, a compound formed by nonpolar covalent bonds, into a glass of water. Instead of instantly dissolving in the water, the oil forms a distinct bead because the polar water molecules repel the nonpolar oil.
Each moment of life, atoms of oxygen, carbon, hydrogen, and the other elements of the human body are making and breaking chemical bonds. Ions are charged atoms that form when an atom donates or accepts one or more negatively charged electrons. Cations (ions with a positive charge) are attracted to anions (ions with a negative charge). This attraction is called an ionic bond. In covalent bonds, the participating atoms do not lose or gain electrons, but share them. Molecules with nonpolar covalent bonds are electrically balanced, and have a linear three-dimensional shape. Molecules with polar covalent bonds have “poles”—regions of weakly positive and negative charge—and have a triangular three-dimensional shape. An atom of oxygen and two atoms of hydrogen form water molecules by means of polar covalent bonds. Hydrogen bonds link hydrogen atoms already participating in polar covalent bonds to anions or electronegative regions of other polar molecules. Hydrogen bonds link water molecules, resulting in the properties of water that are important to living things.
By the end of this section, you will be able to:
One characteristic of a living organism is metabolism, which is the sum total of all of the chemical reactions that go on to maintain that organism’s health and life. The bonding processes you have learned thus far are anabolic chemical reactions; they form larger molecules from smaller molecules or atoms. Recall that metabolism can proceed in another direction: in catabolic chemical reactions, when bonds between components of larger molecules break, releasing smaller molecules or atoms. Both types of reactions involve exchanges not only of matter, but of energy.
The Role of Energy in Chemical Reactions
Chemical reactions require a sufficient amount of energy to cause the matter to collide with enough precision and force that old chemical bonds can be broken and new ones formed. In general, kinetic energy is the form of energy powering any type of matter in motion. Imagine you are building a brick wall. The energy it takes to lift and place one brick atop another is kinetic energy—the energy matter possesses because of its motion. Once the wall is in place, it stores potential energy. Potential energy is the energy of position, or the energy matter possesses because of the positioning or structure of its components. If the brick wall collapses, the stored potential energy is released as kinetic energy when the bricks fall.
In the human body, potential energy is stored in the bonds between atoms and molecules. Chemical energy is the form of potential energy in which energy is stored in chemical bonds. When those bonds are formed, chemical energy is invested, and when they break, chemical energy is released. Notice that chemical energy, like all energy, is neither created nor destroyed, rather, it is converted from one form to another. When you eat an energy bar before heading out the door for a hike, the honey, nuts, and other foods the bar contains are broken down and rearranged by your body into molecules that your muscle cells convert to kinetic energy.
Chemical reactions that release more energy than they absorb are characterized as exergonic. The catabolism of the foods in your energy bar is an example. Some of the chemical energy stored in the bar is absorbed into molecules your body uses for fuel, but some of it is released—for example, as heat. In contrast, chemical reactions that absorb more energy than they release are endergonic. These reactions require energy input and the resulting molecule stores not only the chemical energy in the original components, but also the energy that fueled the reaction. Since energy is neither created nor destroyed, where does the energy needed for endergonic reactions come from? In many cases, it comes from exergonic reactions.
Forms of Energy Important in Human Functioning
You have already learned that chemical energy is absorbed, stored, and released by chemical bonds. In addition to chemical energy, mechanical, radiant, and electrical energy are important in human functioning.
Characteristics of Chemical Reactions
All chemical reactions begin with a reactant, the general term for one or more substances that enter into the reaction. Sodium and chloride ions, for example, are the reactants in the production of table salt. One or more substances produced by a chemical reaction are called the product.
In chemical reactions, the components of the reactants—the elements involved and the number of atoms of each—are all present in the product(s). Similarly, there is nothing present in the products that are not present in the reactants. This is because chemical reactions are governed by the law of conservation of mass, which states that matter cannot be created or destroyed in a chemical reaction.
Just as you can express mathematical calculations in equations such as 2 + 7 = 9, you can use chemical equations to show how reactants become products. As in math, chemical equations proceed from left to right, but instead of an equal sign, they employ an arrow or arrows indicating the direction in which the chemical reaction proceeds. For example, the chemical reaction in which one atom of nitrogen and three atoms of hydrogen produce ammonia would be written as N + 3H→ NH3. Correspondingly, the breakdown of ammonia into its components would be written as NH3 →N + 3H.
Notice that, in the first example, a nitrogen (N) atom and three hydrogen (H) atoms bond to form a compound. This anabolic reaction requires energy, which is then stored within the compound’s bonds. Such reactions are referred to as synthesis reactions. A synthesis reaction is a chemical reaction that results in the synthesis (joining) of components that were formerly separate (Figure 2.3.1a). Again, nitrogen and hydrogen are reactants in a synthesis reaction that yields ammonia as the product. The general equation for a synthesis reaction is A + B→AB.
In the second example, ammonia is catabolized into its smaller components, and the potential energy that had been stored in its bonds is released. Such reactions are referred to as decomposition reactions. A decomposition reaction is a chemical reaction that breaks down or “de-composes” something larger into its constituent parts (see Figure 2.3.1b). The general equation for a decomposition reaction is: AB→A+B.
An exchange reaction is a chemical reaction in which both synthesis and decomposition occur, chemical bonds are both formed and broken, and chemical energy is absorbed, stored, and released (see Figure 2.3.1c). The simplest form of an exchange reaction might be: A+BC→AB+C. Notice that, to produce these products, B and C had to break apart in a decomposition reaction, whereas A and B had to bond in a synthesis reaction. A more complex exchange reaction might be: AB+CD→AC+B. Another example might be: AB+CD→AD+BC.
In theory, any chemical reaction can proceed in either direction under the right conditions. Reactants may synthesize into a product that is later decomposed. Reversibility is also a quality of exchange reactions. For instance, A+BC→AB+C could then reverse to AB+C→A+BC. This reversibility of a chemical reaction is indicated with a double arrow: A+BC⇄AB+C. Still, in the human body, many chemical reactions do proceed in a predictable direction, either one way or the other. You can think of this more predictable path as the path of least resistance because, typically, the alternate direction requires more energy.
Factors Influencing the Rate of Chemical Reactions
If you pour vinegar into baking soda, the reaction is instantaneous; the concoction will bubble and fizz, but many chemical reactions take time. A variety of factors influence the rate of chemical reactions. This section, however, will consider only the most important in human functioning.
Properties of the Reactants
If chemical reactions are to occur quickly, the atoms in the reactants have to have easy access to one another. Thus, the greater the surface area of the reactants, the more readily they will interact. When you pop a cube of cheese into your mouth, you chew it before you swallow it. Among other things, chewing increases the surface area of the food so that digestive chemicals can more easily get at it. As a general rule, gases tend to react faster than liquids or solids, again because it takes energy to separate particles of a substance, and gases by definition already have space between their particles. Similarly, the larger the molecule, the greater the number of total bonds, so reactions involving smaller molecules, with fewer total bonds, would be expected to proceed faster.
In addition, recall that some elements are more reactive than others. Reactions that involve highly reactive elements like hydrogen proceed more quickly than reactions that involve less reactive elements. Reactions involving stable elements like helium are not likely to happen at all.
Temperature
Nearly all chemical reactions occur at a faster rate at higher temperatures. Recall that kinetic energy is the energy of matter in motion. The kinetic energy of subatomic particles increases in response to increases in thermal energy. The higher the temperature, the faster the particles move, and the more likely they are to come in contact and react.
Concentration and Pressure
If just a few people are dancing at a club, they are unlikely to step on each other’s toes. As more and more people get up to dance—especially if the music is fast—collisions are likely to occur. It is the same with chemical reactions: the more particles present within a given space, the more likely those particles are to bump into one another. This means that chemists can speed up chemical reactions not only by increasing the concentration of particles—the number of particles in the space—but also by decreasing the volume of the space, which would correspondingly increase the pressure. If there were 100 dancers in that club, and the manager abruptly moved the party to a room half the size, the concentration of the dancers would double in the new space, and the likelihood of collisions would increase accordingly.
Enzymes and Other Catalysts
For two chemicals in nature to react with each other they first have to come into contact, and this occurs through random collisions. Since heat helps increase the kinetic energy of atoms, ions, and molecules, it promotes their collision. However, in the body, extremely high heat—such as a very high fever—can damage body cells and be life-threatening. On the other hand, normal body temperature is not high enough to promote the chemical reactions that sustain life. That is where catalysts come in.
In chemistry, a catalyst is a substance that increases the rate of a chemical reaction without itself undergoing any change. You can think of a catalyst as a chemical change agent. They help increase the rate and force at which atoms, ions, and molecules collide, thereby increasing the probability that their valence shell electrons will interact.
The most important catalysts in the human body are enzymes. An enzyme is a catalyst composed of protein or ribonucleic acid (RNA), both of which will be discussed later in this chapter. Like all catalysts, enzymes work by lowering the level of energy that needs to be invested in a chemical reaction. A chemical reaction’s activation energy is the “threshold” level of energy needed to break the bonds in the reactants. Once those bonds are broken, new arrangements can form. Without an enzyme to act as a catalyst, a much larger investment of energy is needed to ignite a chemical reaction (Figure 2.3.2).
Enzymes are critical to the body’s healthy functioning. They assist, for example, with the breakdown of food and its conversion to energy. In fact, most of the chemical reactions in the body are facilitated by enzymes.
Chemical reactions, in which chemical bonds are broken and formed, require an initial investment of energy. Kinetic energy, the energy of matter in motion, fuels the collisions of atoms, ions, and molecules that are necessary if their old bonds are to break and new ones to form. All molecules store potential energy, which is released when their bonds are broken.
Four forms of energy essential to human functioning are: chemical energy (which is stored and released as chemical bonds are formed and broken), mechanical energy (which directly powers physical activity), radiant energy (emitted as waves such as in sunlight), and electrical energy, the power of moving electrons.
Chemical reactions begin with reactants and end with products. Synthesis reactions bond reactants together, a process that requires energy, whereas decomposition reactions break the bonds within a reactant and thereby release energy. In exchange reactions, bonds are both broken and formed, and energy is exchanged.
The rate at which chemical reactions occur is influenced by several properties of the reactants: temperature, concentration and pressure, and the presence or absence of a catalyst. An enzyme is a catalytic protein that speeds up chemical reactions in the human body.
By the end of this section, you will be able to:
The concepts you have learned so far in this chapter govern all forms of matter, and would work as a foundation for geology as well as biology. This section of the chapter narrows the focus to the chemistry of human life; that is, the compounds important for the body’s structure and function. In general, these compounds are either inorganic or organic.
The following section examines the four groups of inorganic compounds essential to life: water, salts, acids, and bases. Organic compounds are covered later in the chapter.
Water
As much as 70 percent of an adult’s body weight is water. This water is contained both within the cells and between the cells that make up tissues and organs. Its several roles make water indispensable to human functioning.
Water as a Lubricant and Cushion
Water is a major component of many of the body’s lubricating fluids. Just as oil lubricates the hinge on a door, water in synovial fluid lubricates the actions of body joints, and water in pleural fluid helps the lungs expand and recoil with breathing. Watery fluids help keep food flowing through the digestive tract, and ensure that the movement of adjacent abdominal organs is friction free.
Water also protects cells and organs from physical trauma, cushioning the brain within the skull, for example, and protecting the delicate nerve tissue of the eyes. Water cushions a developing fetus in the mother’s womb as well.
Water as a Heat Sink
A heat sink is a substance or object that absorbs and dissipates heat but does not experience a corresponding increase in temperature. In the body, water absorbs the heat generated by chemical reactions without greatly increasing in temperature. Moreover, when the environmental temperature soars, the water stored in the body helps keep the body cool. This cooling effect happens as warm blood from the body’s core flows to the blood vessels just under the skin and is transferred to the environment. At the same time, sweat glands release warm water in sweat. As the water evaporates into the air, it carries away heat, and then the cooler blood from the periphery circulates back to the body core.
Water as a Component of Liquid Mixtures
A mixture is a combination of two or more substances, each of which maintains its own chemical identity. In other words, the constituent substances are not chemically bonded into a new, larger chemical compound. The concept is easy to imagine if you think of powdery substances such as flour and sugar; when you stir them together in a bowl, they obviously do not bond to form a new compound. The room air you breathe is a gaseous mixture, containing three discrete elements—nitrogen, oxygen, and argon—and one compound, carbon dioxide. There are three types of liquid mixtures, all of which contain water as a key component; these are solutions, colloids, and suspensions.
For cells in the body to survive, they must be kept moist in a water-based liquid called a solution. In chemistry, a liquid solution consists of a solvent that dissolves a substance called a solute. An important characteristic of solutions is that they are homogeneous; that is, the solute molecules are distributed evenly throughout the solution. If you were to stir a teaspoon of sugar into a glass of water, the sugar would dissolve into sugar molecules separated by water molecules. The ratio of sugar to water in the left side of the glass would be the same as the ratio of sugar to water in the right side of the glass. If you were to add more sugar, the ratio of sugar to water would change, but the distribution—provided you had stirred well—would still be even.
Water is considered the “universal solvent” and it is believed that life cannot exist without water because of this. Water is certainly the most abundant solvent in the body; essentially all of the body’s chemical reactions occur among compounds dissolved in water. Since water molecules are polar, with regions of positive and negative electrical charge, water readily dissolves ionic compounds and polar covalent compounds. Such compounds are referred to as hydrophilic, or “water-loving.” As mentioned above, sugar dissolves well in water. This is because sugar molecules contain regions of hydrogen-oxygen polar bonds, making it hydrophilic. Nonpolar molecules, which do not readily dissolve in water, are called hydrophobic, or “water-fearing.”
Concentrations of Solutes
Various mixtures of solutes and water are described in chemistry. The concentration of a given solute is the number of particles of that solute in a given space (oxygen makes up about 21 percent of atmospheric air). In the bloodstream of humans, glucose concentration is usually measured in milligram (mg) per deciliter (dL), and in a healthy adult averages about 100 mg/dL. Another method of measuring the concentration of a solute is by its molarilty—which is moles (M) of the molecules per liter (L). The mole of an element is its atomic weight, while a mole of a compound is the sum of the atomic weights of its components, called the molecular weight. An often-used example is calculating a mole of glucose, with the chemical formula C6H12O6. Using the periodic table, the atomic weight of carbon (C) is 12.011 grams (g), and there are six carbons in glucose, for a total atomic weight of 72.066 g. Doing the same calculations for hydrogen (H) and oxygen (O), the molecular weight equals 180.156g (the “gram molecular weight” of glucose). When water is added to make one liter of solution, you have one mole (1M) of glucose. This is particularly useful in chemistry because of the relationship of moles to “Avogadro’s number.” A mole of any solution has the same number of particles in it: 6.02 × 1023. Many substances in the bloodstream and other tissue of the body are measured in thousandths of a mole, or millimoles (mM).
A colloid is a mixture that is somewhat like a heavy solution. The solute particles consist of tiny clumps of molecules large enough to make the liquid mixture opaque (because the particles are large enough to scatter light). Familiar examples of colloids are milk and cream. In the thyroid glands, the thyroid hormone is stored as a thick protein mixture also called a colloid.
A suspension is a liquid mixture in which a heavier substance is suspended temporarily in a liquid, but over time, settles out. This separation of particles from a suspension is called sedimentation. An example of sedimentation occurs in the blood test that establishes sedimentation rate, or sed rate. The test measures how quickly red blood cells in a test tube settle out of the watery portion of blood (known as plasma) over a set period of time. Rapid sedimentation of blood cells does not normally happen in the healthy body, but aspects of certain diseases can cause blood cells to clump together, and these heavy clumps of blood cells settle to the bottom of the test tube more quickly than do normal blood cells.
The Role of Water in Chemical Reactions
Two types of chemical reactions involve the creation or the consumption of water: dehydration synthesis and hydrolysis.
These reactions are reversible, and play an important role in the chemistry of organic compounds (which will be discussed shortly).
Salts
Recall that salts are formed when ions form ionic bonds. In these reactions, one atom gives up one or more electrons, and thus becomes positively charged, whereas the other accepts one or more electrons and becomes negatively charged. You can now define a salt as a substance that, when dissolved in water, dissociates into ions other than H+ or OH–. This fact is important in distinguishing salts from acids and bases, discussed next.
A typical salt, NaCl, dissociates completely in water (Figure 2.4.2). The positive and negative regions on the water molecule (the hydrogen and oxygen ends respectively) attract the negative chloride and positive sodium ions, pulling them away from each other. Again, whereas nonpolar and polar covalently bonded compounds break apart into molecules in solution, salts dissociate into ions. These ions are electrolytes; they are capable of conducting an electrical current in solution. This property is critical to the function of ions in transmitting nerve impulses and prompting muscle contraction.
Many other salts are important in the body. For example, bile salts produced by the liver help break apart dietary fats, and calcium phosphate salts form the mineral portion of teeth and bones.
Acids and Bases
Acids and bases, like salts, dissociate in water into electrolytes. Acids and bases can very much change the properties of the solutions in which they are dissolved.
Acids
An acid is a substance that releases hydrogen ions (H+) in solution (Figure 2.4.3a). Because an atom of hydrogen has just one proton and one electron, a positively charged hydrogen ion is simply a proton. This solitary proton is highly likely to participate in chemical reactions. Strong acids are compounds that release all of their H+ in solution; that is, they ionize completely. Hydrochloric acid (HCl), which is released from cells in the lining of the stomach, is a strong acid because it releases all of its H+ in the stomach’s watery environment. This strong acid aids in digestion and kills ingested microbes. Weak acids do not ionize completely; that is, some of their hydrogen ions remain bonded within a compound in solution. An example of a weak acid is vinegar, or acetic acid; it is called acetate after it gives up a proton.
Bases
A base is a substance that releases hydroxyl ions (OH–) in solution, or one that accepts H+ already present in solution (see Figure 2.4.3b). The hydroxyl ions (also known as hydroxide ions) or other basic substances combine with H+ present to form a water molecule, thereby removing H+ and reducing the solution’s acidity. Strong bases release most or all of their hydroxyl ions; weak bases release only some hydroxyl ions or absorb only a few H+. Food mixed with hydrochloric acid from the stomach would burn the small intestine (the next portion of the digestive tract after the stomach), if it were not for the release of bicarbonate (HCO3–), a weak base that attracts H+. Bicarbonate accepts some of the H+ protons, thereby reducing the acidity of the solution.
The Concept of pH
The relative acidity or alkalinity of a solution can be indicated by its pH. A solution’s pH is the negative, base-10 logarithm of the hydrogen ion (H+) concentration of the solution. As an example, a pH 4 solution has an H+ concentration that is ten times greater than that of a pH 5 solution. That is, a solution with a pH of 4 is ten times more acidic than a solution with a pH of 5. The concept of pH will begin to make more sense when you study the pH scale, as shown in Figure 2.4.4. The scale consists of a series of increments ranging from 0 to 14. A solution with a pH of 7 is considered neutral—neither acidic nor basic. Pure water has a pH of 7. The lower the number below 7, the more acidic the solution, or the greater the concentration of H+. The concentration of hydrogen ions at each pH value is 10 times different than the next pH. For instance, a pH value of 4 corresponds to a proton concentration of 10–4 M, or 0.0001M, while a pH value of 5 corresponds to a proton concentration of 10–5 M, or 0.00001M. The higher the number above 7, the more basic (alkaline) the solution, or the lower the concentration of H+. Human urine, for example, is ten times more acidic than pure water, and HCl is 10,000,000 times more acidic than water.
Buffers
The pH of human blood normally ranges from 7.35 to 7.45, although it is typically identified as pH 7.4. At this slightly basic pH, blood can reduce the acidity resulting from the carbon dioxide (CO2) constantly being released into the bloodstream by the trillions of cells in the body. Homeostatic mechanisms (along with exhaling CO2 while breathing) normally keep the pH of blood within this narrow range. This is critical, because fluctuations—either too acidic or too alkaline—can lead to life-threatening disorders.
All cells of the body depend on homeostatic regulation of acid–base balance at a pH of approximately 7.4. The body therefore has several mechanisms for this regulation, involving breathing, the excretion of chemicals in urine, and the internal release of chemicals collectively called buffers into body fluids. A buffer is a solution of a weak acid and its conjugate base. A buffer can neutralize small amounts of acids or bases in body fluids. For example, if there is even a slight decrease below 7.35 in the pH of a bodily fluid, the buffer in the fluid—in this case, acting as a weak base—will bind the excess hydrogen ions. In contrast, if pH rises above 7.45, the buffer will act as a weak acid and contribute hydrogen ions.
Homeostatic Imbalances
The excessive acidity of acids and bases, of the blood, and other body fluids is known as acidosis. Common causes of acidosis are situations and disorders that reduce the effectiveness of breathing, especially the person’s ability to exhale fully, which causes a buildup of CO2 (and H+) in the bloodstream. Acidosis can also be caused by metabolic problems that reduce the level or function of buffers that act as bases, or that promote the production of acids. For instance, with severe diarrhea, too much bicarbonate can be lost from the body, allowing acids to build up in body fluids. In people with poorly managed diabetes (ineffective regulation of blood sugar), acids called ketones are produced as a form of body fuel. These can build up in the blood, causing a serious condition called diabetic ketoacidosis. Kidney failure, liver failure, heart failure, cancer, and other disorders also can prompt metabolic acidosis.
In contrast, alkalosis is a condition in which the blood and other body fluids are too alkaline (basic). As with acidosis, respiratory disorders are a major cause; however, in respiratory alkalosis, carbon dioxide levels fall too low. Lung disease, aspirin overdose, shock, and ordinary anxiety can cause respiratory alkalosis, which reduces the normal concentration of H+.
Metabolic alkalosis often results from prolonged, severe vomiting, which causes a loss of hydrogen and chloride ions (as components of HCl). Medications can also prompt alkalosis. These include diuretics that cause the body to lose potassium ions, as well as antacids when taken in excessive amounts, for instance by someone with persistent heartburn or an ulcer.
Inorganic compounds essential to human functioning include water, salts, acids, and bases. These compounds are inorganic; that is, they do not contain both hydrogen and carbon. Water is a lubricant and cushion, a heat sink, a component of liquid mixtures, a byproduct of dehydration synthesis reactions, and a reactant in hydrolysis reactions. Salts are compounds that, when dissolved in water, dissociate into ions other than H+ or OH–. In contrast, acids release H+ in solution, making it more acidic. Bases accept H+, thereby making the solution more alkaline (caustic).
The pH of any solution is its relative concentration of H+. A solution with pH 7 is neutral. Solutions with pH below 7 are acids, and solutions with pH above 7 are bases. A change in a single digit on the pH scale (e.g., from 7 to 8) represents a ten-fold increase or decrease in the concentration of H+. In a healthy adult, the pH of blood ranges from 7.35 to 7.45. Homeostatic control mechanisms that are important for keeping blood in a healthy pH range include chemicals called buffers, weak acids and weak bases released when the pH of blood or other body fluids fluctuates in either direction outside of this normal range.
By the end of this section, you will be able to:
Organic compounds typically consist of groups of carbon atoms covalently bonded to hydrogen, usually oxygen, and often other elements as well. Created by living things, they are found throughout the world, in soils and seas, commercial products, and every cell of the human body. The four types most important to human structure and function are: carbohydrates, lipids, proteins, and nucleotides. Before exploring these compounds, you need to first understand the chemistry of carbon.
The Chemistry of Carbon
What makes organic compounds ubiquitous is the chemistry of their carbon core. Recall that carbon atoms have four electrons in their valence shell, and that the octet rule dictates that atoms tend to react in such a way as to complete their valence shell with eight electrons. Carbon atoms do not complete their valence shells by donating or accepting four electrons. Instead, they readily share electrons via covalent bonds.
Normally, carbon atoms share with other carbon atoms, often forming a long carbon chain referred to as a carbon skeleton. When they share, however, they do not share all their electrons exclusively with each other. Rather, carbon atoms tend to share electrons with a variety of other elements, one of which is always hydrogen. Carbon and hydrogen groupings are called hydrocarbons. If you study the figures of organic compounds in the remainder of this chapter, you will see several with chains of hydrocarbons in one region of the compound.
Many combinations are possible to fill carbon’s four “vacancies.” Carbon may share electrons with oxygen or nitrogen or other atoms in a particular region of an organic compound. Moreover, the atoms to which carbon atoms bond may also be part of a functional group. A functional group is a group of atoms linked by strong covalent bonds and tend to function in chemical reactions as a single unit. You can think of functional groups as tightly knit “cliques” whose members are unlikely to be parted. Five functional groups are important in human physiology: the hydroxyl, carboxyl, amino, methyl and phosphate groups (Table 2.1).
Functional Group | StructuralFormula | Importance |
Hydroxyl | —O—H | Hydroxyl groups are polar. They are components of all four types of organic compounds discussed in this chapter. They are involved in dehydration synthesis and hydrolysis reactions. |
Carboxyl | O—C—OH | Carboxyl groups are found within fatty acids, amino acids, and many other acids. |
Amino | —N—H2 | Amino groups are found within amino acids, the building blocks of proteins. |
Methyl | —C—H3 | Methyl groups are found within amino acids. |
Phosphate | —P—O42– | Phosphate groups are found within phospholipids and nucleotides. |
Carbon’s affinity for covalent bonding means that many distinct and relatively stable organic molecules readily form larger, more complex molecules. Any large molecule is referred to as macromolecule (macro- = “large”), and the organic compounds in this section all fit this description. However, some macromolecules are made up of several “copies” of single units called monomer (mono- = “one”; -mer = “part”). Like beads in a long necklace, these monomers link by covalent bonds to form long polymers (poly- = “many”). There are many examples of monomers and polymers among the organic compounds.
Monomers form polymers by engaging in dehydration synthesis (see Figure 2.4.1). As was noted earlier, this reaction results in the release of a molecule of water. Each monomer contributes; one gives up a hydrogen atom and the other gives up a hydroxyl group. Polymers are split into monomers by hydrolysis (-lysis = “rupture”). The bonds between their monomers are broken, via the donation of a molecule of water, which contributes a hydrogen atom to one monomer and a hydroxyl group to the other.
Carbohydrates
The term carbohydrate means “hydrated carbon.” Recall that the root hydro- indicates water. A carbohydrate is a molecule composed of carbon, hydrogen, and oxygen; in most carbohydrates, hydrogen and oxygen are found in the same two-to-one relative proportions they have in water. In fact, the chemical formula for a “generic” molecule of carbohydrate is (CH2O)n.
Carbohydrates are referred to as saccharides, a word meaning “sugars.” Three forms are important in the body: monosaccharides, disaccharides, and polysaccharides. Monosaccharides are the monomers of carbohydrates. Disaccharides (di- = “two”) are made up of two monomers. Polysaccharides are the polymers, and can consist of hundreds to thousands of monomers.
Monosaccharides
A monosaccharide is a monomer of carbohydrates. Five monosaccharides are important in the body. Three of these are the hexose sugars, so called because they each contain six atoms of carbon. These are glucose, fructose, and galactose, shown in Figure 2.5.1a. The remaining monosaccharides are the two pentose sugars, each of which contains five atoms of carbon. They are ribose and deoxyribose, shown in Figure 2.5.1b.
Disaccharides
A disaccharide is a pair of monosaccharides. Disaccharides are formed via dehydration synthesis, and the bond linking them is referred to as a glycosidic bond (glyco- = “sugar”). Three disaccharides (shown in Figure 2.5.2) are important to humans. These are sucrose, commonly referred to as table sugar, lactose, or milk sugar, and maltose, or malt sugar. As you can tell from their common names, you consume these in your diet, however, your body cannot use them directly. Instead, in the digestive tract, they are split into their component monosaccharides via hydrolysis.
Polysaccharides
Polysaccharides can contain a few to a thousand or more monosaccharides. Three are important to the body (Figure 2.5.3):
Functions of Carbohydrates
The body obtains carbohydrates from plant-based foods. Grains, fruits, and legumes and other vegetables provide most of the carbohydrate in the human diet, although lactose is found in dairy products.
Although most body cells can break down other organic compounds for fuel, all body cells can use glucose. Moreover, nerve cells (neurons) in the brain, spinal cord, and through the peripheral nervous system, as well as red blood cells, can only use glucose for fuel. In the breakdown of glucose for energy, molecules of adenosine triphosphate, better known as ATP, are produced. Adenosine triphosphate (ATP) is composed of a ribose sugar, an adenine base, and three phosphate groups. ATP releases free energy when its phosphate bonds are broken, and thus supplies ready energy to the cell. More ATP is produced in the presence of oxygen (O2) than in pathways that do not use oxygen. The overall reaction for the conversion of the energy in glucose to energy stored in ATP can be written:
C6H12O6 + 6 O2 → 6 CO2 + 6 H2O + ATP
In addition to being a critical fuel source, carbohydrates are present in very small amounts in cells’ structure. For instance, some carbohydrate molecules bind with proteins to produce glycoproteins, and others combine with lipids to produce glycolipids, both of which are found in the membrane that encloses the contents of body cells.
Lipids
A lipid is one of a highly diverse group of compounds made up mostly of hydrocarbons. The few oxygen atoms they contain are often at the periphery of the molecule. Their nonpolar hydrocarbons make all lipids hydrophobic. In water, lipids do not form a true solution, but they may form an emulsion, which is the term for a mixture of solutions that do not mix well.
Triglycerides
A triglyceride is one of the most common dietary lipid groups, and the type found most abundantly in body tissues. This compound, which is commonly referred to as a fat, is formed from the synthesis of two types of molecules (Figure 2.5.4):
Triglycerides form via dehydration synthesis. Glycerol gives up hydrogen atoms from its hydroxyl groups at each bond, and the carboxyl group on each fatty acid chain gives up a hydroxyl group. A total of three water molecules are thereby released.
Fatty acid chains that have no double carbon bonds anywhere along their length and therefore contain the maximum number of hydrogen atoms are called saturated fatty acids. These straight, rigid chains pack tightly together and are solid or semi-solid at room temperature (Figure 2.5.5a). Butter and lard are examples, as is the fat found on a steak or in your own body. In contrast, fatty acids with one double carbon bond are kinked at that bond (Figure 2.5.5b). These monounsaturated fatty acids are therefore unable to pack together tightly, and are liquid at room temperature. Polyunsaturated fatty acids contain two or more double carbon bonds, and are also liquid at room temperature. Plant oils such as olive oil typically contain both mono- and polyunsaturated fatty acids.
Whereas a diet high in saturated fatty acids increases the risk of heart disease, a diet high in unsaturated fatty acids is thought to reduce the risk. This is especially true for the omega-3 unsaturated fatty acids found in cold-water fish such as salmon. These fatty acids have their first double carbon bond at the third hydrocarbon from the methyl group (referred to as the omega end of the molecule).
Finally, trans fatty acids found in some processed foods, including some stick and tub margarines, are thought to be even more harmful to the heart and blood vessels than saturated fatty acids. Trans fats are created from unsaturated fatty acids (such as corn oil) when chemically treated to produce partially hydrogenated fats.
As a group, triglycerides are a major fuel source for the body. When you are resting or asleep, a majority of the energy used to keep you alive is derived from triglycerides stored in your fat (adipose) tissues. Triglycerides also fuel long, slow physical activity such as gardening or hiking, and contribute a modest percentage of energy for vigorous physical activity. Dietary fat also assists the absorption and transport of the nonpolar fat-soluble vitamins A, D, E, and K. Additionally, stored body fat protects and cushions the body’s bones and internal organs, and acts as insulation to retain body heat.
Fatty acids are also components of glycolipids, which are sugar-fat compounds found in the cell membrane. Lipoproteins are compounds in which the hydrophobic triglycerides are packaged in protein envelopes for transport in body fluids.
Phospholipids
As its name suggests, a phospholipid is a bond between the glycerol component of a lipid and a phosphorous molecule. In fact, phospholipids are similar in structure to triglycerides. However, instead of having three fatty acids, a phospholipid is generated from a diglyceride, a glycerol with just two fatty acid chains (Figure 2.5.6). The third binding site on the glycerol is taken up by the phosphate group, which in turn is attached to a polar “head” region of the molecule. Recall that triglycerides are nonpolar and hydrophobic. This still holds for the fatty acid portion of a phospholipid compound. However, the head of a phospholipid contains charges on the phosphate groups, as well as on the nitrogen atom. These charges make the phospholipid head hydrophilic. Therefore, phospholipids are said to have hydrophobic tails, containing the neutral fatty acids, hydrophilic heads, the charged phosphate groups, and nitrogen atom.
Steroids
A steroid compound (referred to as a sterol) has as its foundation a set of four hydrocarbon rings bonded to a variety of other atoms and molecules (see Figure 2.5.6b). Although both plants and animals synthesize sterols, the type that makes the most important contribution to human structure and function is cholesterol, which is synthesized by the liver in humans and animals and is also present in most animal-based foods. Like other lipids, cholesterol’s hydrocarbons make it hydrophobic, however, it has a polar hydroxyl head that is hydrophilic. Cholesterol is an important component of bile acids and compounds that help emulsify dietary fats. In fact, the word’s root chole- refers to bile. Cholesterol is also a building block of many hormones, signaling molecules that the body releases to regulate processes at distant sites. Finally, like phospholipids, cholesterol molecules are found in the cell membrane, where their hydrophobic and hydrophilic regions help regulate the flow of substances into and out of the cell.
Prostaglandins
Like a hormone, a prostaglandin is one of a group of signaling molecules, but prostaglandins are derived from unsaturated fatty acids (see Figure 2.5.6c). One reason that the omega-3 fatty acids found in fish are beneficial is that they stimulate the production of certain prostaglandins that help regulate aspects of blood pressure and inflammation, and thereby reduce the risk for heart disease. Prostaglandins also sensitize nerves to pain. One class of pain-relieving medications called nonsteroidal anti-inflammatory drugs (NSAIDs) works by reducing the effects of prostaglandins.
Proteins
You might associate proteins with muscle tissue, but in fact, proteins are critical components of all tissues and organs. A protein is an organic molecule composed of amino acids linked by peptide bonds. Proteins include the keratin in the epidermis of skin that protects underlying tissues, and the collagen found in the dermis of skin, in bones, and in the meninges that cover the brain and spinal cord. Proteins are also components of many of the body’s functional chemicals, including digestive enzymes in the digestive tract, antibodies, the neurotransmitters that neurons use to communicate with other cells, and the peptide-based hormones that regulate certain body functions (for instance, growth hormone). While carbohydrates and lipids are composed of hydrocarbons and oxygen, all proteins also contain nitrogen (N), and many contain sulfur (S), in addition to carbon, hydrogen, and oxygen.
Microstructure of Proteins
Proteins are polymers made up of nitrogen-containing monomers called amino acids. An amino acid is a molecule composed of an amino group and a carboxyl group, together with a variable side chain. Just 20 different amino acids contribute to nearly all of the thousands of different proteins important in human structure and function. Body proteins contain a unique combination of a few dozen to a few hundred of these 20 amino acid monomers. All 20 of these amino acids share a similar structure (Figure 2.5.7). All consist of a central carbon atom to which the following are bonded:
Notice that all amino acids contain both an acid (the carboxyl group) and a base (the amino group) (amine = “nitrogen-containing”). For this reason, they make excellent buffers, helping the body regulate acid–base balance. What distinguishes the 20 amino acids from one another is their variable group, which is referred to as a side chain or an R-group. This group can vary in size and can be polar or nonpolar, giving each amino acid its unique characteristics. For example, the side chains of two amino acids—cysteine and methionine—contain sulfur. Sulfur does not readily participate in hydrogen bonds, whereas all other amino acids do. This variation influences the way that proteins containing cysteine and methionine are assembled.
Amino acids join via dehydration synthesis to form protein polymers (Figure 2.5.8). The unique bond holding amino acids together is called a peptide bond. A peptide bond is a covalent bond between two amino acids that is formed by dehydration synthesis. A peptide, in fact, is a very short chain of amino acids. Strands containing fewer than about 100 amino acids are generally referred to as polypeptides rather than proteins.
The body is able to synthesize most of the amino acids from components of other molecules, however, nine cannot be synthesized and have to be consumed in the diet. These are known as the essential amino acids.
Free amino acids available for protein construction are said to reside in the amino acid pool within cells. Structures within cells use these amino acids when assembling proteins. If a particular essential amino acid is not available in sufficient quantities in the amino acid pool, however, synthesis of proteins containing it can slow or even cease.
Shape of Proteins
Just as a fork cannot be used to eat soup and a spoon cannot be used to spear meat, a protein’s shape is essential to its function. A protein’s shape is determined, most fundamentally, by the sequence of amino acids of which it is made (Figure 2.5.9a). The sequence is called the primary structure of the protein.
Although some polypeptides exist as linear chains, most are twisted or folded into more complex secondary structures that form when bonding occurs between amino acids with different properties at different regions of the polypeptide. The most common secondary structure is a spiral called an alpha-helix. If you were to take a length of string and simply twist it into a spiral, it would not hold the shape. Similarly, a strand of amino acids could not maintain a stable spiral shape without the help of hydrogen bonds, which create bridges between different regions of the same strand (see Figure 2.5.9b). Less commonly, a polypeptide chain can form a beta-pleated sheet, in which hydrogen bonds form bridges between different regions of a single polypeptide that has folded back upon itself, or between two or more adjacent polypeptide chains.
The secondary structure of proteins further folds into a compact three-dimensional shape, referred to as the protein’s tertiary structure (see Figure 2.5.9c). In this configuration, amino acids that had been very distant in the primary chain can be brought quite close via hydrogen bonds or, in proteins containing cysteine, via disulfide bonds. A disulfide bond is a covalent bond between sulfur atoms in a polypeptide. Often, two or more separate polypeptides bond to form an even larger protein with a quaternary structure (see Figure 2.5.9d). The polypeptide subunits forming a quaternary structure can be identical or different. For instance, hemoglobin, the protein found in red blood cells is composed of four tertiary polypeptides, two of which are called alpha chains and two of which are called beta chains.
When they are exposed to extreme heat, acids, bases, and certain other substances, proteins will denature. Denaturation is a change in the structure of a molecule through physical or chemical means. Denatured proteins lose their functional shape and are no longer able to carry out their jobs. An everyday example of protein denaturation is the curdling of milk when acidic lemon juice is added.
The contribution of the shape of a protein to its function can hardly be exaggerated. For example, the long, slender shape of protein strands that make up muscle tissue is essential to their ability to contract (shorten) and relax (lengthen). As another example, bones contain long threads of a protein called collagen that acts as scaffolding upon which bone minerals are deposited. These elongated proteins, called fibrous proteins, are strong and durable and typically hydrophobic.
In contrast, globular proteins are globes or spheres that tend to be highly reactive and are hydrophilic. The hemoglobin proteins packed into red blood cells are an example (see Figure 2.59d), however, globular proteins are abundant throughout the body, playing critical roles in most body functions. Enzymes, introduced earlier as protein catalysts, are examples of this. The next section takes a closer look at the action of enzymes.
Proteins Function as Enzymes
If you were trying to type a paper, and every time you hit a key on your laptop there was a delay of six or seven minutes before you got a response, you would probably get a new laptop. In a similar way, without enzymes to catalyze chemical reactions, the human body would be nonfunctional. It functions only because enzymes function.
Enzymatic reactions—chemical reactions catalyzed by enzymes—begin when substrates bind to the enzyme. A substrate is a reactant in an enzymatic reaction. This occurs on regions of the enzyme known as active sites (Figure 2.5.10). Any given enzyme catalyzes just one type of chemical reaction. This characteristic, called specificity, is due to the fact that a substrate with a particular shape and electrical charge can bind only to an active site corresponding to that substrate.
Binding of a substrate produces an enzyme–substrate complex. It is likely that enzymes speed up chemical reactions in part because the enzyme–substrate complex undergoes a set of temporary and reversible changes that cause the substrates to be oriented toward each other in an optimal position to facilitate their interaction. This promotes increased reaction speed. The enzyme then releases the product(s), and resumes its original shape. The enzyme is then free to engage in the process again, and will do so as long as substrate remains.
Other Functions of Proteins
Advertisements for protein bars, powders, and shakes all say that protein is important in building, repairing, and maintaining muscle tissue, but the truth is that proteins contribute to all body tissues, from the skin to the brain cells. Also, certain proteins act as hormones and chemical messengers that help regulate body functions. For example, growth hormone is important for skeletal growth, among other roles.
As was noted earlier, the basic and acidic components enable proteins to function as buffers in maintaining acid–base balance, but they also help regulate fluid–electrolyte balance. Proteins attract fluid, and a healthy concentration of proteins in the blood, the cells, and the spaces between cells helps ensure a balance of fluids in these various “compartments.” Moreover, proteins in the cell membrane help to transport electrolytes in and out of the cell, keeping these ions in a healthy balance. Like lipids, proteins can bind with carbohydrates. They can thereby produce glycoproteins or proteoglycans, both of which have many functions in the body.
The body can use proteins for energy when carbohydrate and fat intake is inadequate, and stores of glycogen and adipose tissue become depleted. However, since there is no storage site for protein except functional tissues, using protein for energy causes tissue breakdown and results in body wasting.
Nucleotides
The fourth type of organic compound important to human structure and function are the nucleotides (Figure 2.5.11). A nucleotide is one of a class of organic compounds composed of three subunits:
Nucleotides can be assembled into nucleic acids (DNA or RNA) or the energy compound adenosine triphosphate.
Nucleic Acids
The nucleic acids differ in their type of pentose sugar. Deoxyribonucleic acid (DNA) is nucleotide that stores genetic information. DNA contains deoxyribose (so-called because it has one less atom of oxygen than ribose) plus one phosphate group and one nitrogen-containing base. The “choices” of base for DNA are adenine, cytosine, guanine, and thymine. Ribonucleic acid (RNA) is a ribose-containing nucleotide that helps manifest the genetic code as protein. RNA contains ribose, one phosphate group, and one nitrogen-containing base, but the “choices” of base for RNA are adenine, cytosine, guanine, and uracil.
The nitrogen-containing bases adenine and guanine are classified as purines. A purine is a nitrogen-containing molecule with a double ring structure, which accommodates several nitrogen atoms. The bases cytosine, thymine (found in DNA only) and uracil (found in RNA only) are pyramidines. A pyramidine is a nitrogen-containing base with a single ring structure
Bonds formed by dehydration synthesis between the pentose sugar of one nucleic acid monomer and the phosphate group of another form a “backbone,” from which the components’ nitrogen-containing bases protrude. In DNA, two such backbones attach at their protruding bases via hydrogen bonds. These twist to form a shape known as a double helix (Figure 2.5.12). The sequence of nitrogen-containing bases within a strand of DNA form the genes that act as a molecular code instructing cells in the assembly of amino acids into proteins. Humans have almost 22,000 genes in their DNA, locked up in the 46 chromosomes inside the nucleus of each cell (except red blood cells which lose their nuclei during development). These genes carry the genetic code to build one’s body, and are unique for each individual except identical twins.
In contrast, RNA consists of a single strand of sugar-phosphate backbone studded with bases. Messenger RNA (mRNA) is created during protein synthesis to carry the genetic instructions from the DNA to the cell’s protein manufacturing plants in the cytoplasm and the ribosomes.
Adenosine Triphosphate
The nucleotide adenosine triphosphate (ATP), is composed of a ribose sugar, an adenine base, and three phosphate groups (Figure 2.5.13). ATP is classified as a high energy compound because the two covalent bonds linking its three phosphates store a significant amount of potential energy. In the body, the energy released from these high energy bonds helps fuel the body’s activities, from muscle contraction to the transport of substances in and out of cells to anabolic chemical reactions.
When a phosphate group is cleaved from ATP, the products are adenosine diphosphate (ADP) and inorganic phosphate (Pi). This hydrolysis reaction can be written:
ATP + H2O → ADP + Pi + energy
Removal of a second phosphate leaves adenosine monophosphate (AMP) and two phosphate groups. Again, these reactions also liberate the energy that had been stored in the phosphate-phosphate bonds. They are reversible, too, as when ADP undergoes phosphorylation. Phosphorylation is the addition of a phosphate group to an organic compound, in this case, resulting in ATP. In such cases, the same level of energy that had been released during hydrolysis must be reinvested to power dehydration synthesis.
Cells can also transfer a phosphate group from ATP to another organic compound. For example, when glucose first enters a cell, a phosphate group is transferred from ATP, forming glucose phosphate (C6H12O6—P) and ADP. Once glucose is phosphorylated in this way, it can be stored as glycogen or metabolized for immediate energy.
Organic compounds essential to human functioning include carbohydrates, lipids, proteins, and nucleotides. These compounds are said to be organic because they contain both carbon and hydrogen. Carbon atoms in organic compounds readily share electrons with hydrogen and other atoms, usually oxygen, and sometimes nitrogen. Carbon atoms also may bond with one or more functional groups such as carboxyls, hydroxyls, aminos, or phosphates. Monomers are single units of organic compounds. They bond by dehydration synthesis to form polymers, which can in turn be broken by hydrolysis.
Carbohydrate compounds provide essential body fuel. Their structural forms include monosaccharides such as glucose, disaccharides such as lactose, and polysaccharides, including starches (polymers of glucose), glycogen (the storage form of glucose), and fiber. All body cells can use glucose for fuel. It is converted via an oxidation-reduction reaction to ATP.
Lipids are hydrophobic compounds that provide body fuel and are important components of many biological compounds. Triglycerides are the most abundant lipid in the body, and are composed of a glycerol backbone attached to three fatty acid chains. Phospholipids are compounds composed of a diglyceride with a phosphate group attached at the molecule’s head. The result is a molecule with polar and nonpolar regions. Steroids are lipids formed of four hydrocarbon rings. The most important is cholesterol. Prostaglandins are signaling molecules derived from unsaturated fatty acids.
Proteins are critical components of all body tissues. They are made up of monomers called amino acids, which contain nitrogen, joined by peptide bonds. Protein shape is critical to its function. Most body proteins are globular. An example is enzymes, which catalyze chemical reactions.
Nucleotides are compounds with three building blocks: one or more phosphate groups, a pentose sugar, and a nitrogen-containing base. DNA and RNA are nucleic acids that function in protein synthesis. ATP is the body’s fundamental molecule of energy transfer. Removal or addition of phosphates releases or invests energy.